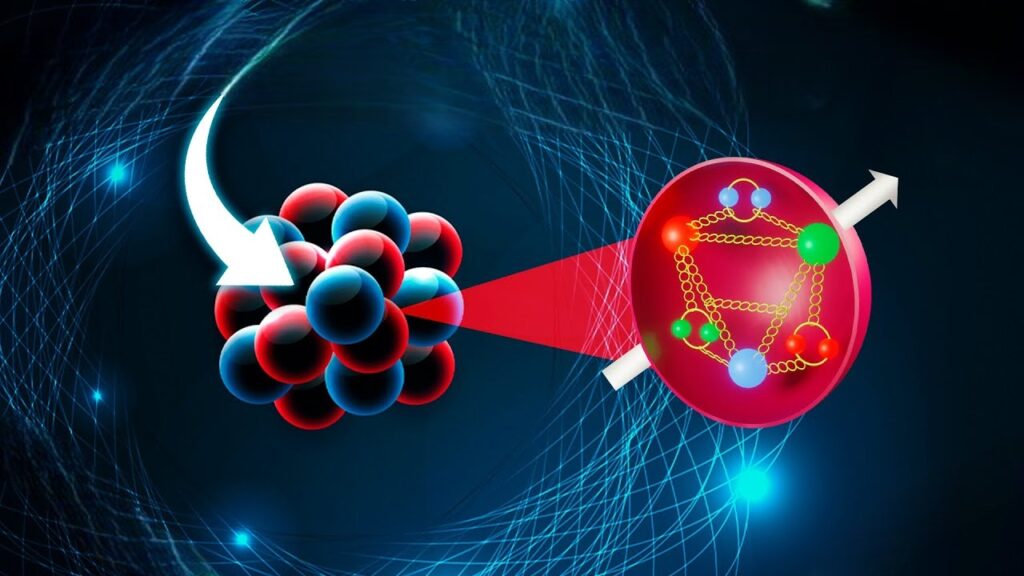
In an atomic nucleus, two protons are electromagnetically repelling one another with enough force to lift a medium-sized labradoodle off the ground.
When this energy is released, a nuclear explosion occurs.
It’s a good thing that our nuclei are held together by something even more powerful than electromagnetism.
However, it is not the powerful force that you might have envisioned.
Certainly not directly. We wouldn’t have complex atoms, chemistry, or even labradoodles if it weren’t for a natural phenomenon that holds nuclei together.
Although the term “quantum chromodynamics” implies a lot of complexity, the fundamental force of nature it refers to—the “strong force”—is actually even more intricate than the name suggests.
However, we left it there.
According to what we learned in that episode, the strong force is only present in nucleons, but it is also the force that binds protons and neutrons together to form the majority of atomic nuclei.
Because it was precisely this question—how are atomic nuclei held together—that led to the discovery of both the strong and weak nuclear forces in the first place, that process is sufficiently odd to merit its own episode.
However, this is also the story of the meson, a mysterious relative of the proton and neutron that, despite being less well-known, is just as essential to the universe’s complex matter.
Hideki Yukawa, a young physicist who graduated from the university of Osaka in 1929, is the protagonist of our narrative. Following his graduation, he moved back in with his parents and began working as a university assistant for no pay.
not because he wasn’t a great physicist, but because of the Great Depression.
Yukawa was concentrating on the issue of how nucleons stuck together all the time.
He was perplexed as to why such a powerful force couldn’t be seen outside of atomic nuclei.
Beta decay, the tendency of large nuclei to occasionally spit out an electron and, as a result, convert one of their neutrons into a proton, provided Yukawa with an intriguing clue to the inner lives of nuclei.
He was aware that photon exchange is how the electromagnetic force is transmitted, so it stood to reason that this nuclear force would be mediated by a particle.
What if this particle was the electron, and the exchange of electrons between nucleons caused beta decay?
Like if electrons and protons were playing catch with each other and occasionally lost the ball.
Although the idea that forces should be mediated by particles was novel at the time, and ultimately led yukawa to the correct response, it turns out that this is completely untrue.
In contrast to the massless photon, creating a short-range force starts with having a mass exchange particle.
We have previously discussed the concept of virtual particles, which are these exchange particles.
In the sense that, as long as they give it back again, virtual particles have the ability to sort of defy the laws of physics by stealing the energy they require for their existence out of thin air.
This is because of the Heisenberg uncertainty principle, which states, in one form, that we will never be able to accurately determine both a phenomenon’s energy and duration.
This indicates that the amount of energy in a region of space can change very quickly.
Because mass is a form of energy, a virtual particle can temporarily acquire mass from nothing; however, the more mass it possesses, the shorter its lifespan will be.
In the event that this particle is traveling at or near the speed of light, Yukawa used the electron mass to determine the magnitude and range of a force mediated by it.
The resulting force had a range much larger than the size of the atomic nucleus and was estimated to be around 1/200th of the strength required to hold the nucleus together.
Therefore, the electron cannot be the particle acting as a mediator of this nuclear force.
However, Yukawa now had an equation that outlined the desired force.
He changed the equation to describe a force with the right strength and range, and he found that a much bigger exchange particle was needed.
Because it had to have a mass somewhere in between that of an electron and a proton, he gave this hypothetical particle the name “meson,” which comes from the Greek word “mesos,” which means middle.
Additionally, Yukawa predicted that these particles would require an electric charge.
A neutron can release a negative meson and become a proton, a proton can release a positive meson and become a neutron, and the neutral meson can be exchanged between protons or neutrons, just like in beta decay.
Yukawa therefore predicted three different types of mesons, one for each possible charge value.
Unfortunately, beta decay was not even partially explained by this new theory.
In point of fact, Yukawa reasoned that beta decay necessitated a distinct nuclear force that was significantly less powerful than the one that held the nucleus together.
In point of fact, he correctly predicted the simultaneous occurrence of the strong and weak forces.
Despite the fact that electrons do not actually mediate the weak force.
In 1935, Yukawa published his theory, which was almost entirely ignored.
To be fair, claiming the existence of two new natural forces was an extraordinary claim that necessitates extraordinary proof.
Until we found a meson, nobody would believe this.
Particle accelerators allow us to regularly smash atoms together to produce a large number of mesons.
However, since particle accelerators were still in their infancy at the time, we were forced to rely on cosmic rays.
Natural space particle accelerators like supernovae and quasars release these high-energy particles onto Earth.
In the hope of inspiring other researchers to look for mesons, Yukawa wrote a letter to nature magazine explaining why cosmic radiation could contain them. However, his letter was still rejected because other researchers had independently considered looking for new particles in cosmic radiation.
For instance, we have bibha chowdhuri and debendra mohan bose, who invented a method that made use of photographic plates.
Because our atmosphere is so good at blocking cosmic rays, they set these up at high altitudes in India and Tibet, which is great for anyone else but annoying for particle physicists.
In addition, chowdhuri and bose found the meson precisely at the mass yukawa predicted.
Unfortunately, most of their work went unnoticed until British scientists independently discovered mesons in 1947.
In any case, these new particles not only had the right mass but also had three of them, one positive, one negative, and one neutral, as predicted by Yukawa.
But things started to get awkward.
More and more mesons with a variety of properties were discovered over the next few years as a result of improved particle accelerators and a better understanding of radiation.
The particles that protons and neutrons actually exchange in the nucleus are referred to as pions, and they are the first mesons that were discovered by Bose and Chowduri.
However, there were many more, including kaons, eta mesons, d mesons, and b mesons, among others.
Even worse, researchers discovered additional particles that, like protons and neutrons, could “exchange mesons.”
The Greek word for “heavy” inspired the name given to these particles, baryons.
The lambda, sigma, xi, and omega were among the others.
Again, each with distinct spins, charges, and masses.
The particle zoo was a huge physics problem because of the interminable collection of baryons and mesons.
The atomic nucleus only requires two baryons—the proton and neutron—and three mesons—the pions, according to yukawa’s theory.
So, what was the point of all this extra junk that nature doesn’t seem to use other than particle accelerators for?
Murray Gell-Mann, who discovered that mesons and baryons are made of quarks rather than elementary particles, was the one who ultimately solved this issue.
A particle that is made up of multiple quarks is referred to as a hadron.
The particle zoo is simply the result of all possible quark combinations.
This was covered in our episode on quantum chromodynamics, but before we get to the punchline of this video, here is a quick refresher.
With the discovery of quarks, it became abundantly clear that the strong force was needed to keep the nucleons and the nucleus together.
In point of fact, the strong force really is the latter.
Nucleon binding is somewhat of an afterthought.
The strong force works on things with color charge, just as electromagnetism works on things with electric charge.
In contrast to the one type of electric charge, there are three color charges.
For instance, a quark could have a charge of red, green, or blue, or it could have a charge of the opposite color, such as antired, antigreen, or antiblue.
Moreover, these names are merely analogies, of course.
The three quark baryons have one of each color charge that cancels each other out, while the two quarks of a meson will have a color and its anti-color. Composite particles must be color neutral.
The gluon, a massless particle, mediates the strong force that holds these quarks together.
When quarks are absorbed, the colors change due to gluons, which carry one regular and one anti-color charge.
A constant exchange of virtual gluons binds mesons and baryons together.
Wait a minute; haven’t we always maintained that the mass-transmitted mesons that carry the strong force account for its short range?
That was Yukawa’s entire argument, but it turns out that mesons aren’t the strong force’s actual exchange particles.
And the gluons, the real ones, lack mass?
What’s happening?
In point of fact, the color-neutral hadrons do not even exert an electrostatic force on each other, just as electrically neutral objects do not feel the strong force directly.
However, there is a solution.
From a distance, atoms appear to be electrically neutral, but when you get close enough to the nucleus, you can feel the positive electric charge.
Similar to this, the internal quarks of protons or neutrons will sense each other’s presence if they are sufficiently close to one another.
A quark from a neutron in close proximity, for example, will try to pull at a quark from a proton.
However, they are unable to do so by simply exchanging a gluon because gluons have color charge, and exchanging a gluon would render the hadrons color neutral, which is against the rules.
Hadrons require the exchange of a neutral particle in order to properly experience the powerful force.
And nature has come up with a way to accomplish that.
How does it work?
A quark from one of the nucleons is pulled toward the other when they get close to each other.
It generates two new quarks when its gluon connection to the other quarks in its nucleus, which we refer to as a flux tube, both extends and snaps.
One stays in the nucleus, while the other joins the original escaped quark to form a quark-antiquark pair.
Our pion, or meson, is here.
Because it doesn’t change color, the second nucleus can absorb it without breaking any physics.
There, an antiquark counterpart in that nucleus and one of the meson’s quarks collide.
Two nucleons of the same type as when we started are all that remain, but they have now communicated the strong force.
Even though they did it in a strange way, the process still exchanged energy and momentum, bringing the particles together.
The strong force circumvents its lack of a neutral gluon in this manner.
The meson, a neutral exchange particle, is put together by it.
However, the meson is mass.
Because a virtual meson can’t exist for very long because it needs a lot of energy to borrow, the uncertainty principle says it can’t.
The possible size of an atomic nucleus is defined by its range.
A nucleus will decay if it grows too big because its nucleons are too far apart to effectively exchange mesons.
Moreover, the residual strong force between nucleons is referred to as the strong nuclear force, whereas the force between quarks is referred to as the strong force.
This brings to mind our episode on quasi particles, you know.
In that episode, we discussed how fields can emerge as emergent behaviors of other fields, with their own quasiforces and quasiparticles, and how these quasifields can arise.
In a way, that’s what’s going on here.
The meson is a quasi-force that acts as a mediator for the strong nuclear force.
Hydrogen would be the most complex atom in the universe if this minuscule quirk of nature—the emergence of a force that is not really intended to be there—did not occur.
Fortunately, nature discovered a way to bind the nuclei, which gave us chemistry, biology, and ourselves. A young physicist named Hideki Yukawa discovered some of the most significant forces from which this richly complex spacetime emerges.
Explore More: